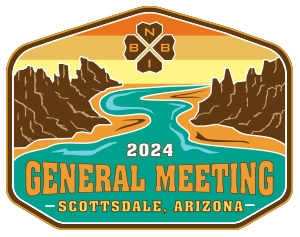
92nd General Meeting Presentation
Beyond the Headlines: A Path to Practical Fusion Power
David Rasmussen
The following remarks were delivered at the General Session of the 92nd General Meeting on May 13, 2024. It has been edited for content and phrasing.
INTRODUCTION: David Rasmussen has been a plasma research scientist at Oak Ridge National Laboratory since 1981 and is the senior advisor to the US International Thermonuclear Experimental Reactor (US ITER) project, which is responsible for providing US contributions to build the ITER tokamak fusion device, a 35-nation collaboration currently being assembled in France. Rasmussen’s areas of research and responsibility have included fusion plasma heating and fueling systems. He has over 40 years of fusion and plasma science experience in plasma measurements and the technology development needed for magnetic confinement fusion and other plasma technology applications. Rasmussen received a Ph.D. in applied science, specializing in plasma physics, from the University of California at Davis, where his graduate work was a study of inertial laser fusion and wave plasma interactions.
His slide presentation can be found here.
MR. RASMUSSEN: I want to thank the National Board for allowing me to give this presentation and Mark Lower (program manager at Oak Ridge) for talking them into it, I think. I was asked to talk about the headlines. There are a lot of headlines in fusion, so I'm only going to talk about them a little bit and go beyond that.
First, I’d like to do a little bit on fusion basics, understanding some of the fusion news that's out there, those announcements you see, and then go into the challenges for fusion. We have pressure vessels. We’ll talk about the fusion environment, the high-precision components, and three main technical challenges that I think are important to this community. Then, we'll discuss current public and private activities in fusion. There's a lot of new activity there.
Of course, we have the sun. It's a very impressive fusion reactor as our oldest star in the universe. Many of you saw the eclipse, where you could see some of these solar flares. And then I think many more of you probably were able to see the effects of those solar flares in the aurora that amazingly came down as far south as Tennessee, where I live. I certainly never thought I'd see them from Tennessee.
Our goal and our fusion research are to make a reactor that confined of hot plasma, like the sun, by heating hydrogen atoms and the isotopes of hydrogen. With that, it's possible to create an artificial star and, with the help of this community, produce abundant energy that can be converted into electricity.
Here’s a little bit on the basics. There are two main approaches to fusion energy that we could talk about. One is inertial fusion. That's the top left image. That's the work you see with compression, lasers, or other directed energy that implode very small pellets of deuterium and tritium. The other is net fusion, which is done at a much lower particle density in large devices and vacuum vessels, surrounded by large magnets that create a toroidal magnetic field. And that toroidal magnetic field in the current plasma itself is the basis for what actually confines the plasma. We're not confining it with metal walls; we're confining it with magnetic fields.
The reaction that is the easiest one in the universe is the deuterium-tritium. It's illustrated in the right-hand diagram. When deuterium and tritium come together for a very brief period, they make a combined atom, which quickly breaks up into helium. We call it an alpha particle because it doesn't have its electron attached to it when it's born from the reaction – and free neutrons.
With the split in energy, 20% goes into that alpha particle helium nucleus at very high energy, three-and-a-half MeV. Then, the neutron comes up with 14 MeV. In most fission reactors, energies for the neutrons are closer to one or one and a half MeV.
What we make use of is the fact that when we're doing magnetic fusion or when we're doing inertial fusion, that alpha particle is available to heat our plasma. I'll show some examples of both cases. The fact that it can come back and do fusion self-heating means we can reduce the total amount of external power we put in that orange arrow that's at the bottom left.
If we can get the external heating energy well below the heating we get from the self-heating, then we'll get a net gain. And we'll do two things with the neutrons. The neutrons primarily will deposit their energy in some thick layer of material using lithium. That lithium will eventually get through a cycle that ultimately results in steam. But some of the neutrons convert that lithium into tritium. And tritium is one of the fuels that we need. We need deuterium, which is quite easy to get from natural sources, but we need to breed our own tritium.
Overall, if you get to the point where that orange arrow says heat, you're into a traditional cycle of making steam and making electricity. The fuel, we like to say, is plentiful worldwide. And it's in every water on earth; it's in seawater; it's in Coca-Cola; and it's relatively easy to extract. As I said before, we have to produce tritium as part of our reactor scheme. The fuel supply can last millions of years. It's hard to imagine how long it can last. It is intrinsically safe and operates at quite high temperature, but the amount of fuel in the vessel at any given time is consumed in less than a second. You can easily turn off the fusion reactor. I've spent most of my career trying to turn them on.
The National Academy has a study discussing the challenges. It gives credit for the class of physics that we've worked on for so many years. We've demonstrated fusion on what they call a small scale, which is appropriate. Then there's just a list of engineering challenges, so this is really what I want to talk about today with you.
But first, here is a little bit about the headlines. You no doubt would have seen some announcements from Lawrence Livermore National Laboratory back in 2022, and then they repeated them in 2023. What they were able to accomplish, you see in the bottom left picture, is their target, which they call a hohlraum. You can see a person is holding it in their hand. It's in a chamber like the one shown just to the right of that. It's quite a large chamber, but it's a vacuum chamber. And then this huge array of laser equipment. They're parallel laser beams of very high intensity focused on that tiny little hohlraum. Inside the hohlraum is a very small fuel pellet that has the deuterium and tritium in it.
What they accomplished is something they've been trying to achieve for quite a while, and they have worked with physics and the understanding of the lasers and the timing of the lasers. The image at the top right is on the scale of the purple circle before the blue image is a little over 100 micrometers. If I were to lay a human hair across there, it would basically cover that spot; it's an extremely small focal area.
When they drove with the laser beams and the X-rays generated in that hohlraum, they were able to compress that pellet to densities that are a thousand times liquid density. And in that region, they're seeing images of the neutrons coming out of that spot. And they demonstrated that they could put in about two megajoules of energy and get a little over three megajoules out.
The other approach is magnetic fusion. Until recently, the world's largest operating tokamak was the JET tokamak in the UK. It’s a very large vacuum vessel. In the bottom left picture, you can see the human-sized, orange structures of the magnets themselves and then the stainless steel vacuum chamber. They operated it in late 2023 and were able to generate 69 megajoules of sustained fusion energy for six seconds. That sounds kind of short. It's an amazingly long time for us who have been working in magnetic fusion, but I want to show you where we're headed with that in a little bit.
In the meantime, you're going to see press announcements from fusion machines all around the world. There's the very large tokamak that will be in Japan, and it will be the largest in the world. There are two very large tokamaks in Korea and one in China.
There's something called a stellarator, which is kind of a cousin of a tokamak, operating in Germany. All have achieved some of their goals toward the ultimate operation of their machines. You’ll see their press releases, usually having to do with the high temperature, but sometimes with some other aspect of the technology.
The project that I'm most involved in is what we call ITER. It has an English acronym, but it doesn't have an acronym in 35 countries so we simply call it ITER. Fortunately, ITER also means in Latin "the way," so we're pretty happy with that as the title.
This is the full plant being built in France with all the countries listed there: the European Union is the host, China, India, Japan, Korea, Russia, and the United States. Much like the space program, we've been cooperating with the Russians and other countries on fusion for longer than the space program – back to the mid-1950s.
What are the challenges? You can imagine the future fusion plant that looks a bit like the one I showed you, the JET with the plasma inside it, doughnut-shaped plasma, humming along, making lots of neutrons, and self-heating itself. It will have all these magnets and electromagnets around it. It will have the area where we're depositing the energy from the neutrons of the lithium. Then, there will be a power conversion system, as it's labeled here, but basically, a water-cooling circuit that flows through the hot lithium that's been raised in temperature by the neutrons. And then out of that will come electricity.
Here are some numbers. A coal plant that many of you might be familiar with makes a little over 1,000 megawatts of electricity using 90 railcars of coal a day. A magnetic fusion plant operating at the same levels and getting 1,000 megawatts of electricity will use a pound of deuterium a day and a pound-and-a-half of lithium. Some of that lithium gets converted to the tritium that we need. It will make two-and-a-half pounds of helium plus 1,000 megawatts of electricity. That's the promise.
ITER is designed to go a long way toward that promise. It's supposed to get a gain of 10. We'll put in 50 megawatts of power and get 500 megawatts of power out of it. It's designed to run for up to 3,000 seconds. Most of the experiments we do will probably be around 400 seconds. We'll test this deuterium-tritium fuel cycle. We'll test industrial-scale fusion systems and integration and operation. We also have to go through a licensing process where the licensee will be the French government and their ASM.
Here's a little more of a picture of the facility so you can see it from the top view, but it is an R&D facility. We're not going to take that power and put it on the grid because it will only operate for up to an hour, and then we’ll be off for a while. We don't operate 24/7. It's still an experiment, but you'll learn a lot about operations, plasma scenarios, and the configurations of our equipment. Long durations will understand the operational issues. We have a lot of instruments that will go on it that will help us learn much more about how to measure and enhance the performance.
The one I want to get to is the cooling part. The United States is responsible for delivering 9% of the pumps for ITER. Part of our 9% is that enormous water-cooling system on the left side. If you follow all those pipes down to the bottom left, there's one of these so-called blanket components; it’s basically a three-layer tile. The back of it is stainless steel with all the water-cooling channels. Some of those channels are in the copper plate that's attached, and then there is a very thin layer of tungsten on top of the copper. It's the tungsten that gets the fluence of the particles. All those structures get the fluence of the neutrons because they're streaming right through those structures. Most of them go through some interaction. Then the water-cooling system keeps the temperature of those tiles on the backside a little over 200 degrees Celsius, but on the front side, they're probably going to be around 600 degrees Celsius on the very surface. We need a lot of conventional things that you guys are used to, such as pressurized tanks and condenser cores. We provide a long list of pumps and various things for this project. We're under PED regulations in Europe.
To give you the scale of what we're building, the left-hand picture shows a portion of the vacuum vessel that will be made up of nine segments that look like that. Of course, they'll be stood up on their end and welded together into the torus. They did a temporary installation on one of them in the main hall where we're going to build a tokamak. You can see it there with a worker coming down the side. They've attached the electromagnets on both sides of that vacuum vessel sector. It will have 18 electromagnets that are nested around the vacuum vessel.
There's an enormous cryogenics plant – I think it's the largest ever built. It's a little hard to see the scale there unless you find some stair steps or things, but it's primarily used to cool our electromagnets. We also use it for other purposes as part of our fuel cycle. It's up and has been commissioned up to its acceptance criteria.
I now want to discuss how we will capture the heat from our new confluence. The little image to the left shows you a very thick layer of lithium. All that green would be a lithium blanket. Some of it is incorporated into those tiles up front. Each of those tiles will have to have essentially a heat exchanger inside it with counterflowing lithium, either solid lithium or counterflowing lithium, and then counterflowing coolant of some other kind. We're talking about either water or helium. Most people like the idea of helium. I don't really want to run against lithium.
The right-hand side of it gives you more of an exploded view, but anyway, you can see how something like that might be put together. But we're not there yet. We're just making cartoons and testing various things. But the disciplines we need are the ones listed on the left-hand side.
Here’s a little bit about the challenge of what those materials have to handle if you go to the sun's surface – not where there's a solar flare. You'd give out one megawatt of power coming at you per meter squared. Our power at the edge of our tokamak will be 10 megawatts per square unit when fully operated. And we're running at a temperature that's more than 10 times the temperature of the sun. We're nowhere near as dense as the sun, but we're 10 times hotter.
The complicated chart on the right side lists on the bottom axis the number of displacements per atom from the neutron flux. The fusion would have to operate in a region where you would have 150 displacements per atom during the lifetime. Some of the more modern designs for fission reactors, Generation IV, for instance, are up in that category, so the challenges of fusion and fission overlap.
The community is growing because of an influx of interest, particularly interest backed by investment. The Department of Energy has stepped up, and the White House announced its bold decadal vision for fusion. The National Academy has an infused program that we use to interface between the national laboratories and the fusion energy community. Several different national labs are listed. And at the bottom is the Fusion Industry Association, which is full of private companies. They are self-organized and cooperate with one another. And they cooperate with the public parts of the project as well. It doesn't mean there aren't some rough edges because we all have to get to know each other and work in our own self-interest, but there are a lot of common problems to solve. You'll see companies, a lot of them in the US, as well in Canada and Europe, and Japan, have a few $6 billion in global private investment. There are a variety of fusion approaches. The ones shown have to do with magnetic fusion, but all these devices have teams of people who work very hard to get these concepts to work across the US. The UK is quite involved as well.
To summarize, several areas must be addressed before we can make electricity with fusion. One of those is supply chain development. Pretty much everything we build now is one-offs or a few-offs. We have a pretty big workforce for plasma physics, not so much for the technology we have to build, so we need workforce development. Those of you who are young out there, we need you.
We need to demonstrate economic viability. We don't know what the cost of electricity will be. We have our estimates, but they are estimates at this point. We need safety and regulatory certainty. There are people in this meeting who are working on code development. We have good interactions with the NRC and others talking about how to treat fusion in some aspects like fission and in other ways not like fission. And eventually, we will have utilities integrated into the market.
One last comment: I'm really glad to be here. I'm learning a lot. You guys are teaching me things I certainly didn't know much about. Engineers who work for me certainly know the codes, they apply the codes, and they demonstrate that their equipment is safe, but it's really quite nice for me to see how you guys develop the codes and enforce them. Well, thank you.
Questions?
MEMBER: How long do you think before there's any kind of – are we talking like …
MR. RASMUSSEN: Electricity on the grid?
MEMBER: Yeah.
MR. RASMUSSEN: That's something that's in active discussion, I would say. The private companies are telling their investors that there's a fairly short horizon for them – 10 years, maybe 15 years. At ITER, we have a longer horizon than that. We're a much larger device than most of them are building. And, again, it's an experiment so that we won't put power on the grid. The next step back for ITER is for all those countries that are involved to go off and build their own fusion power plants, so we have visions for all those things.
MEMBER: Obviously, we're at the very beginning here, so is this to be considered a return on investment?
MR. RASMUSSEN: Yes.
MEMBER: But if we were to look at the percentage of what we're putting in versus what we're getting out as far as any value to the grid, what would we be looking at? It's still theoretical; a lot of it is.
MR. RASMUSSEN: The driver is, like many things, filled with capital costs, how long the plant lives, and how much maintenance you have to do. It's those walls that I described. They are the thing that will wear out the quickest. And they're the things that will need the most maintenance. There’s a very active study on how to develop and get good properties for those materials. You have to look at replacement rates that are something like 10 years. The lifetime of the reactor is 40-plus years. The fuel is quite expensive. The amount of lithium we need initially in the blanket is a significant investment. We consume tiny amounts, but you'll have to buy that initial lithium.
MEMBER: Besides the deuterium, tritium, and lithium, are there any other byproducts, like hazardous ones, that we're currently producing in our traditional reactors?
MR. RASMUSSEN: The materials that it's made out of will have neutrons flowing through them, so they get activated. It's not a product, but it is a waste stream. When you would go to decommission a plant, you would have a waste stream of activated components. We design them or will design them as we go forward to have relatively short half-lives, not 100,000-year half-lives, but 100-year half-lives so that they're not huge. Many of them will have much shorter half-lives, so we're not generating a huge inventory of activated materials that have to be watched through 100,000 years.
MEMBER: Maybe they would generally be in the building you'd have to deal with. You're not making a continuous supply.
MR. RASMUSSEN: No, we don't have any byproducts other than the helium, which, of course, you can release.
MEMBER: Were there any carryover or companion technologies with a large matter of concern that they're working together?
MR. RASMUSSEN: Because both use cryogenics or magnets, there was a big overlap. They also mostly divert a bit of the beam that diverges outward to activate things, so some of those things are electronics. We learned a lot from them about what electronics to choose for our control systems and how to qualify them, so yes, there has been a transfer of knowledge. That, again, is an experiment. It's from pure experiment.
MEMBER: Is there a target for the net cost for kilowatt hour?
MR. RASMUSSEN: The target is what's out there in the market already, so it competes with all the other or will compete with all the other technologies that might be around in that time frame, so solar, wind, and fission. If you don't meet those costs of electricity, then we won't do it. Thank you.